Summary¶
Adopting soil and crop management practices that conserve or enhance soil structure is critical for supporting the sustainable adaptation of EU agriculture to climate change, as it should help maintain agricultural production in the face of increasing drought or water excess without impairing environmental quality. In this chapter, we evaluate the evidence for this assertion by synthesizing the results of 36 published meta-analyses of the effects of soil and crop management practices on soil physical and hydraulic properties and hydrological processes relevant for climate change adaptation in European agriculture. We also review an additional 127 meta-analyses that investigated synergies and trade-offs or help to explain effects of soil and crop management in terms of the underlying processes and mechanisms. We also identify, as far as possible, how responses to alternative soil-crop management systems vary under contrasting agro-environmental conditions (soil type, climates) across the EU. This information may help practitioners and policymakers to draw context-specific conclusions concerning the efficacy of management practices as climate adaptation tools.
Our synthesis demonstrates that organic soil amendments and the adoption of cropping systems and practices that maintain “continuous living cover” result in significant beneficial effects for the water regulation function of soils, mostly arising from the additional carbon inputs to soil and the stimulation of biological processes. These effects are clearly related to improved soil aggregation and enhanced bio-porosity, both of which reduce surface runoff and increase infiltration. One potentially negative consequence of systems that maintain “continuous living cover” is a reduction in soil water storage and groundwater recharge, which may be problematic in dry climates. No other significant trade-offs are known, while some important synergies have been identified including, for example, reductions in nitrate leaching to groundwater and greenhouse gas emissions for non-leguminous cover crop systems.
The benefits of reducing tillage intensity appear much less clear-cut. Increases in soil bulk density due to traffic compaction are commonly reported. However, biological activity under reduced and no-till practices is enhanced, which should in principle improve soil structure, increase infiltration capacity and reduce surface runoff and the losses of agro-chemicals to surface water. However, the evidence for these beneficial effects is not convincing and some significant trade-offs have also been identified, including yield penalties, increased herbicide use and leaching risks for pesticides and nitrate as well as larger greenhouse gas emissions.
Introduction¶
As a consequence of on-going climate change, the occurrence of extreme weather events, such as high temperatures, summer droughts, waterlogging and flooding, will most probably increase in many parts of Europe (IPCC (2021)) An urgent task is to develop guidance on management practices that help farmers adapt to these extreme weather situations.
The ecosystem services a soil can deliver, and therefore its potential as a climate adaptation tool, depend profoundly on its structure, which we define here as the physical arrangement of the soil pore space. Mediated by various biological (e.g. faunal and microbial activity) and physical processes (e.g. traffic compaction, wet-dry and freeze-thaw cycles), soil structure is constantly evolving at time scales ranging from seconds to centuries, driven by weather patterns as well as changes in climate and land management practices. In turn, soil structure strongly affects all life in soil as well as the abiotic hydrological processes that determine the balance between infiltration, surface runoff, drainage and the retention of water in soil and therefore the supply of water and nutrients to crop plants. Thus, adopting soil and crop management practices that enhance soil structure is a key way to support the sustainable adaptation of EU agriculture to climate change by maintaining agricultural production in the face of increasing drought or water excess, without impairing environmental quality. Some relevant management practices are considered part of conservation agriculture Palm , 2014. Another more recently coined term is regenerative agriculture, which implicitly acknowledges past failures to preserve soil quality or soil health Schreefel , 2020. Conservation agriculture to improve soil structure rests on three fundamental principles Palm , 2014: i.) minimizing mechanical soil disturbance, ii.) maintaining soil cover by plants as much as possible and for as long as possible (i.e. aspects of both spatial and temporal coverage), and iii.) diversifying cropping. Another related term, which focuses specifically on climate adaptation, is “climate-smart agriculture”, defined by FAO (2010) as “… agriculture that sustainably increases productivity, enhances resilience, reduces greenhouse gases, and enhances achievement of national food security and development goals”.
The effects of soil and crop management practices on soil properties, soil hydrological and biological functioning and crop performance have been studied in many long-term field trials throughout the world. In addition to narrative reviews (e.g. Palm (2014)), many quantitative meta-analyses synthesizing the findings of individual experiments have also been published. This is especially the case in the last few years Beillouin , 2019Beillouin , 2019, probably because the number of field experiments that have been running for a sufficient length of time has only recently reached the critical mass required to enable these kinds of quantitative analysis. Indeed, the increase in the number of meta-analyses published on topics related to conservation agriculture has been so dramatic that three over-arching syntheses of these meta-analyses have also recently been published. Two of these studies focused on specific aspects of conservation agriculture, one on the use of organic amendments and cover crops on soil organic matter (SOM) storage Bolinder , 2020)and another on the effects of crop diversification strategies on a range of ecosystem services Beillouin , 2019. In addition, Tamburini (2020) carried out an even more ambitious and comprehensive global review of 98 meta-analyses of the effects of conservation agriculture on a wide range of ecosystem services, including a second-order meta-analysis on 69 of these studies. They concluded that diversification practices most often resulted in a ‘win-win’ situation for ecosystem services including crop yields, but that the often large variability in responses and the occurrence of trade-offs highlighted the need to analyze the context-dependency of outcomes, something which was only possible to do to a limited extent with such a broad-brush treatment. Furthermore, previous syntheses of meta-analyses on the benefits of conservation agriculture have placed very little emphasis Tamburini , 2020 or none at all Beillouin , 2019Bolinder , 2020Beillouin , 2019 on soil hydrological functioning even though this is key for climate change adaptation. In their synthesis, Tamburini (2020) included 17 meta-analyses (involving 31 effects-size comparisons) relevant to water regulation, but most of these concerned water quality issues rather than hydrological functioning per se. Beillouin (2019) concluded that … “our review reveals that a significant knowledge gap remains, in particular regarding water use”.
In this study, we focus on the implications of agricultural management practices for soil hydrological functioning for climate change adaptation, specifically under European agro-environmental conditions. We do this by identifying and synthesizing existing meta-analyses of the response of soil physical/hydraulic properties and hydrological processes relevant for climate change adaptation to soil and crop management practices. Some meta-analyses focused only on the average effect of a given management practice and did not attempt to analyze variations in effects depending on local conditions such as soil type and climate. In those cases where such information is available, we summarize knowledge of context-specific effects of relevance for the range of agro-environmental conditions found within the EU, and as far as possible, explain these variations in terms of individual driving processes and mechanisms. This kind of information may explain local praxis in agricultural management (i.e. farmer choices) and will also enable practitioners and policymakers to draw context-specific conclusions concerning the efficacy of management practices as climate adaptation tools. We also perform analyses of redundancy and sensitivity and assess the quality of the documentation for the studies included in the synthesis. This qualitative evaluation highlights where consensus has been established and also identifies remaining knowledge gaps.
Materials and Methods¶
Literature search¶
A brainstorming exercise within the CLIMASOMA project consortium was first carried out to establish potential keywords to be used in the search string. This exercise consisted in mapping keywords on soil properties/states, processes, external drivers and human drivers related to soil water status, crop water supply and the resilience of agricultural ecosystems. This ensured a systematic approach for literature collection, which would be less biased by our personal previous knowledge and experience. A link to this map is given in the supplementary information. From this map, we developed the search string shown in Figure 1. This search string was used to search the published literature using Web of Knowledge in May 2021. This search returned 663 results. The approach is described and the results listed in the “query” notebook.
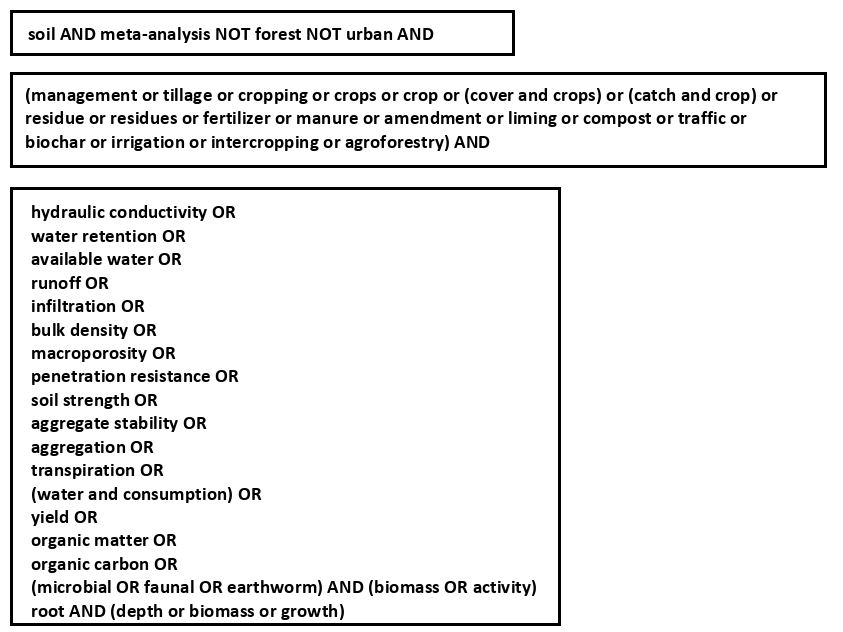
All search results were manually assessed for their relevance to the objectives of our study. Meta-analyses that only included studies carried out outside Europe (i.e. North America or China) were not retained. Our search identified 36 relevant meta-analyses focusing on the effects of soil and crop management on soil physical properties and hydrological processes using effects ratios (Table S1 in the supplementary information, see Supplementary materials Chapter 1 ). Figure 2 shows the number of primary studies per publication year included in the 36 meta-analyses. A peak is clearly visible in 2014, which is explained by the fact that all of the selected meta-analyses were published after 2015. Our search string was also designed to identify meta-analyses of management effects on soil organic matter and biological response variables (e.g. microbial biomass), since these help to explain the observed effects on physical/hydraulic properties and hydrological responses, as well as other studies that analyzed target variables representing potential “trade-offs” and synergies. With respect to the latter, we focused primarily on the impacts of management practices on crop yields, greenhouse gas emissions and water quality. An additional 154 published meta-analyses of this kind were identified by our literature search. These supporting studies are listed in the supplementary information (“Supporting studies.xlsx”).
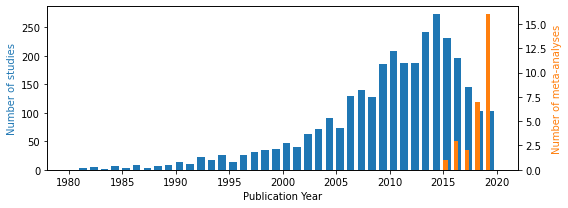
Number of primary studies included in the 36 selected meta-analyses published per year and the publication year of these meta-analyses.
The target variables (i.e. soil physical and hydraulic properties, soil water states) and drivers (i.e. soil/crop management practices) included in the 36 meta-analyses were then classified into a limited number of groups. The target variables were grouped into 5 classes: pore space properties (e.g. porosity, bulk density), hydraulic properties (e.g. saturated hydraulic conductivity, field capacity), mechanical properties (e.g. soil aggregate stability, penetration resistance), water flows (e.g. infiltration, surface runoff, drainage) and plant properties (e.g. root length density, water use efficiency). Similarly, the management practices were grouped into 5 classes: soil amendments (e.g. manure, biochar, organic farming systems), cropping practices and systems (e.g. cover crops, crop rotations), tillage systems (e.g. no-till), grazing management and irrigation. In total, we report 104 treatment comparisons from the 36 meta-analyses that summarize the impacts of drivers on response variables. The relationships (either positive, negative or neutral i.e. non-significant) between drivers and target variables were read from tables and figures in each meta-analysis. Figure 3 summarizes the statistical relationships found between the drivers and target variables in the selected meta-analyses.
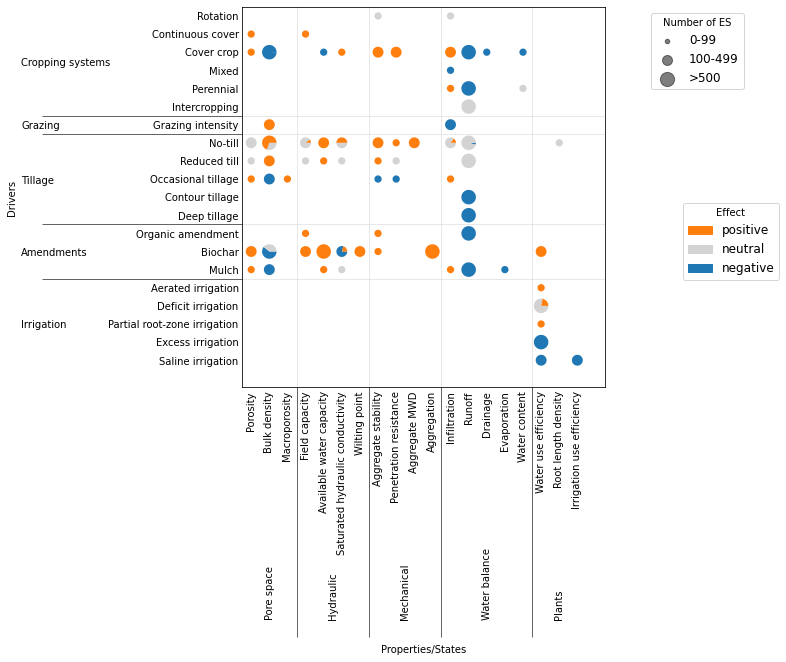
Effects of drivers (vertical axis) on target variables (horizontal axis) in the 36 selected meta-analyses. The coloured pie charts represent the directions of the statistical effects in the different meta-analyses, while the size of the circle indicates the total number of effects sizes (ES) reported. Blank cells denote that no data was available for this target variable in any of the selected meta-analyses.
Gaps in the scatter plots shown in Figure 3 indicate particular combinations of drivers and target variables that have not been the subject of meta-analysis according to our search criteria. Inspection of these figures suggests that there are several significant “knowledge gaps”. Although several meta-analyses have focused on the effects of irrigation management or organic amendments on water use efficiency, almost nothing has been published about the effects of soil management on root growth and water supply to crops, something which is critical for adaptation to future climates with more frequent and severe summer droughts. We can therefore only make inferences about the effects of soil management on crop transpiration from other terms in the soil water balance. Other knowledge gaps may be only apparent and therefore less serious: macroporosity is rarely studied in the context of meta-analysis, although infiltration has been much more frequently measured and these two variables should be strongly correlated. Figure 3 also indicates that some management practices have been less often the subject of field experiments including, for example, deep tillage, occasional tillage, and crop rotations. Presumably for reasons of cost, many long-term field experiments often only have simple designs, neglecting potentially interesting combinations of treatments, for example, no-till combined with the use of cover crops. Similarly, the interactions between soil and crop management and irrigation or drainage systems and practices do not appear to be a common topic of field experimentation.
Some additional potential knowledge gaps concerning the effects of soil management on water regulation functions are not revealed by inspection of Figure 3, since they concern variables that are rarely measured and so have not yet been the subject of meta-analysis. Most long-term field trials on the effects of soil and crop management practices on hydrological and biological functioning have measured surrogate variables (or proxies) for soil structure, such as infiltration rates or soil hydraulic properties (water retention, hydraulic conductivity at and near saturation). No meta-analyses have been performed yet for metrics quantifying different aspects of soil structure per se Rabot , 2018 even though the application of X-ray imaging techniques to quantify soil structure is now becoming increasingly common. As a result, the number of X-ray studies published is rapidly increasing, so it should not be too long before it will be possible and worthwhile to carry out such an analysis.
Quality assessment¶
We performed a quality assessment of the selected 36 meta-analyses using 15 of the criteria proposed by Beillouin , 2019. Figure 4 presents a summary of the quality of the selected meta-analyses according to these criteria. The results for each individual meta-analysis are provided in Table S2 in the supplementary information. The Collaboration for Environmental Evidence (CEEDER) also provides criteria to assess the quality of meta-analysis (Konno (2020); https://environmentalevidence.org/ceeder/). We attempted to use these criteria, but we felt that their complexity (four levels of quality) and the subjectivity of the decision-making made it difficult to assess some of the questions (e.g. ‘Is the search comprehensive?’ or ‘Is the choice of synthesis approach appropriate?’). We therefore preferred to use the simpler yes/no approach of Beillouin , 2019.
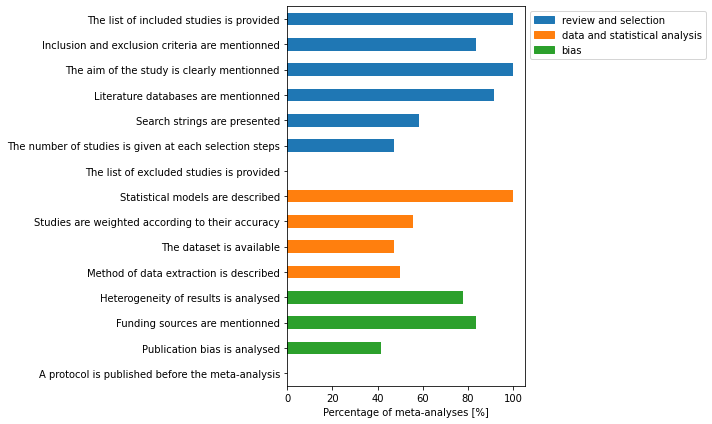
Proportion of the quality criteria defined by Bellouin et al. (2019a) that are met by the selected meta-analyses in this study.
Figure 4 shows that two of the quality criteria were not met by any of the 36 primary meta-analyses included in our study, in that no prior protocol was published and a list of excluded studies was not provided. Only a little over 40% of the meta-analyses included datasets in the paper, while only nine of the meta-analyses (i.e. 32%) investigated the important issue of publication bias Philibert , 2012. The authors of these nine studies used simple statistical techniques such as frequency distributions of effects sizes or “funnel plots” of sample sizes against effect sizes to investigate whether experiments showing non-significant effects are under-represented in the literature. For both of these methods, symmetry of the distributions is taken to indicate a lack of bias. Only two of the nine studies detected any evidence of publication bias Basche & DeLonge, 2019Shackelford , 2019 and in both cases, the effects on the overall conclusions of the studies were thought to be marginal. Several studies also investigated sensitivity of the outcome to the exclusion of individual studies.
Redundancy¶
We performed a redundancy analysis to identify the proportion of common primary or source studies among the meta-analyses following the methodology of Beillouin , 2019. For each of the 36 selected meta-analyses, the references to the studies used were extracted from the supplementary materials. Each reference contained at least the name of the first author, the year of publication, the title, the journal and – if available – the DOI. Of the 3142 unique primary studies, 437 had no DOI. Old publications or publications not written in English were usually found to have no DOI. In some cases, the title and DOI were not available and we had to manually check these references based on contextual information supplied in the supplementary material. For instance, considering the string ‘Mgwango et al. 2011’ in a meta-analysis on tillage and knowing the study took place in ‘Tanzania’ led to a unique reference identification. In most cases, however, the title was provided in the meta-analysis and the DOI could be extracted automatically from the Cross-Ref database. We then manually checked if the title of the paper matched the one found on Cross-ref, to confirm the DOI assignment. Figure 5 shows the redundancy matrix between meta-analyses.
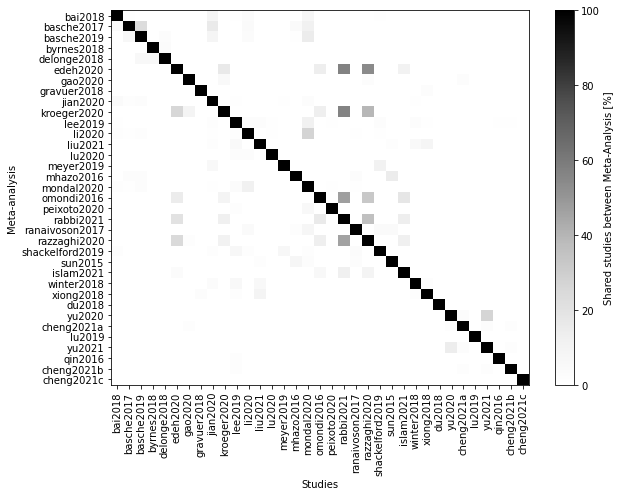
Redundancy matrix showing the percentage of shared studies among meta-analyses. The percentage refers to the number of shared studies divided by the total number of studies in the meta-analysis in the row. Note that this matrix is not symmetrical, because the percentage is computed for the meta-analysis in the row. If we had shown the number of shared studies as a number and not a percentage, this matrix would have been symmetrical.
The notebook redundancy contains the results of this redundancy analysis. Note that for this analysis, the studies of Li et al. (2019) and Li et al. (2020a) were considered as one, as they both rely on the same database, but analyze different variables. First, we identified the studies shared between multiple meta-analyses and computed the percentage of shared studies per meta-analysis. Figure 6 shows the percentage of shared studies (number of shared studies divided by number of studies in the meta-analysis in the row times 100). Figure 6 shows for each meta-analysis the number of source studies that it shares with at least one other meta-analysis. Some meta-analyses share nearly 100% of their studies with another meta-analysis (e.g. Omondi (2016) , Edeh (2020)). In addition to the extent of redundancy, Figure 6 also shows the number of primary studies included in each meta-analysis. For example, Jian (2020), Li et al. (2020a) and Mondal (2020) considered more than 200 primary studies in their meta-analyses. Finally, Figure 7 shows for each meta-analysis the percentage of its primary studies that are shared with another meta-analysis. For example, the studies by Razzaghi (2020) and Rabbi (2021) share a large proportion of primary studies. Figure 6 also shows that nearly all of the primary studies included in these two meta-analyses are shared with another meta-analysis.
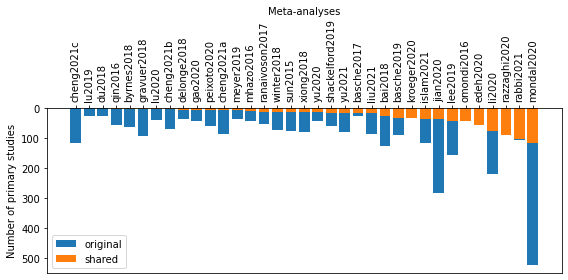
Histogram showing the number of studies per meta-analysis. The studies shared by at least one other meta-analysis are displayed in light green (shared), while the studies found only in this meta-analysis are shown in dark green (original).
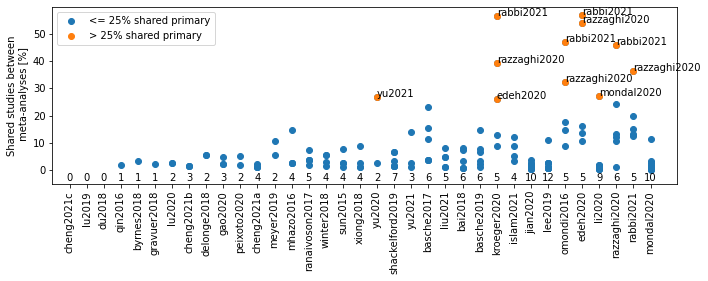
Redundancy among the selected meta-analyses (horizontal axis). Dots represent the percentage of shared primary studies between two meta-analyses. When this percentage is above 25%, the dots are shown in red and the name of the meta-analysis is displayed. For instance, Li (2020) shares more than 25% of its primary studies with the meta-analysis of Mondal (2020). The number on the horizontal axis denotes the number of other meta-analyses that share primary studies with the meta-analysis named horizontally. Note that several meta-analysis do not share any studies with others. Meta-analysis are sorted according to the amount of shared primary studies they have (same order as Figure 6).
Results and discussion¶
Cropping systems and practices¶
Broadly speaking, published meta-analyses that have investigated the effects of cropping systems and practices (Figure 3) fall into two categories: i.) studies analyzing the effects of maintaining a more continuous soil surface cover, either in a temporal (e.g. cover crops in arable rotations) or in a spatial sense (e.g. inter-row cover in widely-spaced row crops such as vineyards and orchards), and ii.) studies comparing farming systems (e.g. continuous arable contrasted with either perennial crops or rotations or mixed farming systems with livestock). In the following, we combine these two aspects, referring to both of them as cropping systems that as far as possible maintain a “continuous living cover” Basche & DeLonge, 2017.
Figure 3 shows that meta-analyses have identified several beneficial effects of such agronomic practices on important physical and hydraulic properties in soil, such as porosity or bulk density, saturated hydraulic conductivity and aggregate stability Basche & DeLonge, 2017Jian , 2020. These positive effects are almost certainly due to a combination of the protective effects of surface cover against the degradation of soil structure by raindrop impact as well as the enhancement of various biological processes that occurs as a consequence of plant growth, root production and the additional carbon supply to the soil. In this respect, meta-analyses have demonstrated that practices that maintain a continuous living cover (e.g. rotations, cover crops) promote increases in microbial biomass, activity and diversity Kim , 2020Muhammad , 2021Jian , 2020Venter , 2016Shackelford , 2019 and increase soil organic matter contents in the long-term Poeplau & Don, 2015King & Blesh, 2018Bai , 2019Bolinder , 2020McClelland , 2021Aguilera , 2013Jian , 2020McDaniel , 2014Shackelford , 2019. This will both promote stable soil aggregation and reduce soil bulk density Meurer , 2020Chenu , 2000Meurer , 2020. The abundance of soil meso- and macro-fauna also increases under long-term cover cropping Reeleder , 2006Roarty , 2017 and perennial crops such as grass/clover leys Fraser , 1994Jarvis , 2017Bertrand , 2015. Through their burrowing activity, these “ecosystem engineers” Jones , 1994 create networks of large biopores in soil Jarvis, 2007 that greatly increase saturated and near-saturated hydraulic conductivity and thus infiltration capacity (e.g. Bertrand (2015); Capowiez (2021)).
The changes in soil physical and hydraulic properties brought about by the introduction of continuous living cover have significant beneficial consequences for the water regulation function of soils. Thus, cover crops enhance infiltration capacity and reduce surface runoff Liu , 2021Basche & DeLonge, 2019Lee , 2019Jian , 2020Xiong , 2018. An increased proportion of perennial crops in the rotation and the presence of ground cover between the rows of perennial crops (e.g. in vineyards) increase soil infiltration and reduce surface runoff Liu , 2021Basche & DeLonge, 2019Xiong , 2018. These positive effects seem broadly similar regardless of climate Liu , 2021Xiong , 2018 .
Some negative consequences of mixed farming systems with grazing livestock for soil physical properties have been noted. Meta-analyses have shown that high grazing intensities result in significantly poorer soil physical quality, in terms of larger bulk densities Byrnes , 2018 and reduced infiltration rates Basche & DeLonge, 2019DeLonge & Basche, 2018 as a result of compaction by animal trampling. These impacts of intensive grazing are similar irrespective of soil texture or climate, although they appear to be slightly larger in wetter climates Byrnes , 2018DeLonge & Basche, 2018. Another significant trade-off is that by increasing transpiration, systems employing “continuous living cover” may reduce soil water content Shackelford , 2019 and decrease recharge to groundwater. Thus, for a combined dataset of 36 studies comprising both experimental and modelling studies, Meyer (2019) found that cover crops reduced recharge by 27 mm/year on average with no apparent effects of climate, soil type or cropping system. For their meta-analysis based on a more limited dataset of six studies, Winter (2018) found no significant effects of inter-row vegetation in vineyards on the soil water balance. Other impacts of cover crops on physical properties with adverse consequences for crop water supply have been reported, in particular an increase in soil penetration resistance and a reduced available water capacity Jian , 2020, although it seems quite difficult to identify plausible mechanisms for such effects. As noted earlier, “continuous living cover” increases soil organic matter contents and both long-term field experiments and meta-analyses suggest that soil organic matter generally tends to increase the plant available water capacity. However, although the magnitude of this effect is still a matter of debate Lal, 2020, in most cases it seems relatively small compared with the crop water demand Minasny & McBratney, 2018Minasny & McBratney, 2018Libohova , 2018 .
With respect to potential synergies and trade-offs, studies have shown that cover crops have either neutral or positive effects on main crop yields Angus , 2015Valkama , 2015Tonitto , 2006Quemada , 2013Marcillo & Miguez, 2017, while non-leguminous cover crop species generally significantly reduce both nitrate leaching and N20 emissions, although this is not the case for legumes Muhammad , 2019Valkama , 2015Tonitto , 2006Basche , 2014Quemada , 2013Shackelford , 2019. Our literature search did not reveal any meta-analyses on phosphorus or pesticide losses.
Tillage systems¶
A large number of meta-analyses have investigated the effects of tillage practices on soil properties and functions and the provision of various ecosystem services (see Figure 3). The control treatment in these published meta-analyses is usually conventional tillage (CT), which involves both inversion ploughing and shallow secondary tillage operations for seedbed preparation. This control treatment is then contrasted with either reduced (or minimum) tillage (RT), whereby the soil is no longer ploughed, or no-till (NT) systems in which the soil is left completely undisturbed, or both. One meta-analysis has investigated the effects of deep tillage and contour tillage on surface runoff Xiong , 2018, while another has analyzed the effects of occasional tillage with no-till as the control treatment Peixoto , 2020.
Reductions in the depth and intensity of tillage (i.e. from CT to RT to NT) strongly influence carbon cycling in soil-crop systems. Meta-analyses show that soil organic carbon concentrations increase under RT and NT systems in the uppermost soil layers (e.g. Bai (2018); Lee (2019)) and especially in fine-textured soils Bai , 2019. The reasons for this are the lack of soil disturbance that promotes a stable aggregated structure, which affords a greater physical protection of C against microbial mineralization Kan , 2021 as well as the elimination of physical mixing and re-distribution of C within the topsoil due to the absence of soil inversion by ploughing Meurer , 2020. Meta-analyses have shown that the accumulation of SOM universally found in surface soil layers under no-till, which reflects the deposition and accumulation of plant residues, is paralleled by a greater microbial biomass compared with CT Li , 2020Lee , 2019Chen , 2020Li , 2018Spurgeon , 2013Li , 2020Zuber & Villamil, 2016. Similarly, enzyme activities are greater under NT Lee , 2019Zuber & Villamil, 2016. In their study, Zuber and Villamil found that NT systems increased β-glucosidase activity, but only close to the soil surface. Increases in enzyme activity under NT should indicate a greater functional microbial diversity. Indeed, meta-analyses have consistently found greater bacterial diversity under conservation tillage. In one early meta-analysis, a significantly larger diversity of fungal communities was also identified Spurgeon , 2013, although later meta-analyses found no such effects Graaff , 2019Li , 2020. Apparent contradictions may be explained by how crop residues are managed, as greater impacts on microbial diversity, including fungal communities, have been found in studies where NT treatments were combined with crop residue retention Li , 2020. We advocate that future studies should always report the combined practices of tillage and residue management, in order to gain further insights into the effects and interactions of tillage and agricultural residue management.
In addition to focusing on organic carbon concentrations in topsoil, differences in SOC stocks under conservation tillage systems in complete crop rooting zones and soil profiles are also of interest, not least from the point of view of climate change mitigation. From their meta-analysis of deeper soil profiles, Mangalassery (2015) concluded that NT systems result in a net sequestration of carbon. This finding appears to contrast with Luo (2010), who had earlier concluded that NT did not increase soil carbon stocks based on a meta-analysis of studies with measurements made to at least 40 cm depth. This is because although SOC is universally larger under NT than CT systems in the uppermost soil layers, it can be significantly smaller both at plough depth and in the upper subsoil (Angers & Eriksen-Hamel (2008)). Thus, for boreo-temperate climates, Haddaway (2017) and Meurer (2018) found increases in soil carbon stocks under NT compared to CT only in the topsoil, while no overall significant effect on carbon stocks was detected for soil profiles to 60 cm depth. In a more recent global meta-analysis, Mondal (2020) found no significant differences in stocks of soil organic carbon between NT and CT systems while variations in response could not be attributed to either climate or soil type. In contrast, Sun (2020) demonstrated significant effects of climate on the potential for carbon sequestration under NT systems. In their global analysis, they found that soil C sequestration was enhanced in warmer and drier regions, while soils under no-till in colder and wetter climates were as likely to lose soil C as gain C. These findings are supported by the regional-scale studies of Meurer (2018) for boreo-temperate climates and González-Sánchez (2012) and Aguilera (2013) for Mediterranean climates, although for vineyards, Payen (2021) found larger topsoil C sequestration in temperate climates than hot and dry climates. A loss of organic carbon following adoption of NT systems is due to a decrease in carbon input resulting from poorer crop growth Pittelkow , 2015Mangalassery , 2015, which compensates for reductions in carbon mineralization rates Virto , 2012Ogle , 2012. Such yield penalties under no-till are especially prevalent in colder and wetter climates Sun , 2020.
Soil tillage directly affects soil macro-fauna by mechanically harming or killing them. In addition to these direct effects of soil disturbance, disruption of the soil also exposes soil macro-fauna to increased risks of desiccation and predation. Consequently, meta-analyses show that total earthworm biomass and abundance increase as tillage intensity is reduced Bai , 2018Spurgeon , 2013Briones & Schmidt, 2017, with a negative relationship between tillage depth and earthworm abundance Briones & Schmidt, 2017. Deep burrowing and surface-feeding (anecic) earthworm species are particularly favoured by NT systems, as their permanent burrows are no longer destroyed by ploughing and they have a better access to food resources. Thus, a lack of disturbance of the soil by tillage has also been shown to increase the diversity of earthworm populations in particular Spurgeon , 2013Briones & Schmidt, 2017Chan, 2001 and soil fauna in general Graaff , 2019.
Changes in tillage systems directly affect the physical properties of soil. For example, bulk density and penetration resistance often increase after the adoption of RT and NT systems Lee , 2019Li , 2020Li , 2019 due to ongoing traffic compaction and the lack of loosening by cultivation Hamza & Anderson, 2005. Peixoto (2020) showed that these negative effects can be alleviated with occasional tillage. The impacts of conservation tillage practices on soil biological agents and processes also give rise to significant indirect effects on physical properties, hydrological processes and ecosystem services related to water regulation. Thus, meta-analyses have shown that saturated hydraulic conductivity and surface infiltration rates often, though not always, increase under conservation tillage compared with CT, especially for NT systems Mondal , 2020Basche & DeLonge, 2019Li , 2020Li , 2019. This suggests that the effects of the enhanced bioporosity in NT systems created by soil fauna, and especially anecic earthworms, on saturated and near-saturated hydraulic conductivity Lee & Foster, 1991 generally outweigh the negative effects of increased bulk density. Thus, Spurgeon (2013) showed in their meta-analysis that increased earthworm abundances and diversity found under NT systems were positively correlated with infiltration rates. Comparing ecological groups, they found that the density of anecic earthworms was positively associated with increased infiltration rates, whereas no effect was apparent for endogeic earthworms. Aggregate stability is also largest under NT systems, decreases when occasional tillage is practiced Peixoto , 2020 and is smallest in CT systems Bai , 2018. In their meta-analysis, Spurgeon (2013) showed that improved aggregate stability under NT systems were positively associated with increases in fungal biomass. A lack of soil disturbance in NT systems also increases the mean size of aggregates produced in stability tests Mondal , 2020Li , 2020. Several meta-analyses have demonstrated increases in field capacity and available water capacity under reduced and no-till systems Mondal , 2020Li , 2020Li , 2019, presumably due to enhanced soil biological activity and increases in organic carbon content. This would improve water supply to crops under drought, although the effects appear relatively small.
In principle, better-developed soil macropore systems and improvements in aggregate stability should promote a more favourable water balance, with increases in infiltration and reductions in surface runoff. Figure 3 shows that the effect on runoff is one of the most studied hydrological processes related to tillage. The meta-analysis performed by Sun (2020) found that reduced and no-till systems decreased surface runoff. However, these results do not appear to be conclusive as two later meta-analyses Xiong , 2018Mhazo , 2016 failed to detect significant effects of conservation tillage practices on surface runoff. However, Xiong et al. found that contour tillage and deep tillage both reduced surface runoff.
Adoption of no-till and reduced till systems involve several trade-offs, particularly concerning water quality, GHG emissions and crop yields. As noted earlier, NT systems tend to give smaller yields for many crops compared with conventional tillage Pittelkow , 2015Sun , 2020Mangalassery , 2015. This may explain why no-till systems are still seldom adopted in Europe Bai , 2018Mangalassery , 2015, although reduced tillage (RT) is being increasingly adopted worldwide. In their comprehensive meta-analysis, Pittlekow et al. identified several reasons for variations in the yield response to no-till. Crop type was the most important, with no significant yield losses found under NT for oilseed, cotton and legume crops, while the yields of cereals and root crops were on average ca. 5% and 20% smaller respectively. In accordance with the results of the meta-analyses on stocks of soil organic carbon discussed earlier, Pittelkow (2015) and Sun (2020) also found climate to be a significant factor, with no significant yield losses for no-till systems under rain-fed conditions in dry climates. In contrast, Peixoto et al. (2020) showed that occasional tillage could increase crop yields in comparison with NT in dry regions and in soils with limited water retention capacity and availability, presumably by alleviating soil compaction and improved rooting.
With respect to water quality, Daryanto (2017) found on an overall 40% reduction in phosphorus loads in surface runoff for NT systems in comparison with CT. This was attributed to significant decreases in losses of particulate phosphorus, as concentrations of dissolved P actually increased in runoff under NT. For pesticides, Elias (2018) found no significant differences in concentrations in surface runoff for 14 of the 18 compounds included in their meta-analysis. Pesticide concentrations were actually larger under NT for the remaining 4 compounds. For loads, no significant difference was detected in between CT and NT systems for 15 of the 18 pesticide compounds. For the three remaining pesticides, losses in surface runoff were larger under NT for metribuzin and dicamba and smaller for alachlor. As also noted by Elias et al., these results seem quite surprising given the documented effects of conservation tillage on soil structure and hydraulic properties in the uppermost soil layers discussed earlier, which should increase soil infiltration capacity and reduce surface runoff. For nitrate losses in surface runoff in conventional and no-till systems, Daryanto (2017) showed that a change to NT resulted in an increase of nitrate concentrations in surface runoff, but similar loads, implying that surface runoff was, as expected, less prevalent under NT.
Daryanto (2017) also performed a meta-analysis on nitrate leaching. They found larger leachate losses of nitrate under NT systems than CT, whereas the concentrations in leachate were similar under both tillage systems, indicating that the effect of NT on nitrate leaching was largely determined by increases in water percolation. No meta-analyses on the effects of tillage systems on pesticide leaching were uncovered by our literature search. Leaching is the outcome of several interacting processes involving many complex and poorly understood trade-offs Alletto , 2010. In practice, with no mechanical disturbance, larger quantities of pesticides are often used to control weeds and diseases in NT systems. However, pesticide leaching will also be highly sensitive to changes induced by tillage in soil structure, microbial biomass and activity and SOC, since these will affect water flow velocities, degradation rates and the strength of adsorption in soil. Several field studies suggest that the better-preserved macropore networks established under RT and NT systems may enhance leaching by preferential flow Jarvis, 2007Alletto , 2010Larsbo , 2009. Although it is difficult to draw firm conclusions about the effects of conservation tillage practices on pesticide leaching without the help of quantitative meta-analyses, we may tentatively conclude that the greater risk of macropore flow under RT and NT systems appears to outweigh any beneficial impacts of increases in SOC and microbial activity on pesticide adsorption and degradation.
Significant trade-offs have also been reported with respect to greenhouse gases. In an early meta-analysis, van Kessel (2013) found no overall impact of reduced tillage or no-till on N20 emissions, with observed increases in humid climates compensated by reductions in emissions in drier climates, although neither trend was significant. However, in a later meta-analysis, Mei (2018) reported a significant overall increase of 18% in N20 emissions under conservation tillage, with the largest effects in warmer and wetter climates and in finer-textured soils. In a recent meta-analysis, Shakoor (2021) found significant increases of emissions of C02, N20 and CH4 of 7, 12 and 21% respectively under NT compared with CT. From the perspective of mitigating climate change, Guenet (2021) concluded that increased emissions of greenhouse gases under no-till outweighed any minor gains in soil C storage.
Amendments¶
Biochar¶
Biochar is charcoal made for the purpose of soil amendment. It is a type of black carbon, resulting from incomplete combustion of organic matter through a process known as pyrolysis. Apart from its potential for long-term soil carbon sequestration, it also has beneficial effects on nutrient availability and soil physical properties Joseph , 2021. Schmidt (2021) reviewed 26 global meta-analyses published between 2016 and 2020 with a focus on the effects of biochar on agronomic performance. Some of the meta-analyses and some of the variables reported in our study were also included in their study. However, in our review we included a larger number of physical properties and two meta-analyses published in 2021.
We included seven meta-analyses on the effects of biochar on hydraulic properties in our review Edeh , 2020Omondi , 2016Kroeger , 2021Razzaghi , 2020Gao , 2020Rabbi , 2021Islam , 2021. These analyses contain data from different soil types, textural classes and experimental conditions (i.e. field or laboratory/greenhouse study). Furthermore, biochars with different properties were applied at different rates. One of these studies Kroeger , 2021 used multiple regression to evaluate the effects of these parameters while the others calculated mean effects for all data or data in sub-categories. Two of the seven meta-analyses only presented data in sub-categories (e.g. for different types of biochar) and did not present results for the overall effects of biochar addition. Nevertheless these analyses do show that biochar has several positive effects on soil hydraulic properties, but that these effects are dependent on all of the above-mentioned variables.
One out of four meta-analyses reported decreased bulk density after biochar addition and one reported no effect while the overall effect was not reported in the remaining studies (see Figure 3). Porosity increased in both meta-analyses where it was included. The density of biochar is low and the porosity is often high compared to soil, which may explain the observed effects. However, if biochar mainly fills existing pores, porosity will decrease and bulk density increase. Biochar will also influence these variables indirectly through its effects on aggregation Pituello , 2018.
Four studies included effects of biochar addition on the water content at field capacity (θfc; pressure potentials in the range -0.033—0.01 MPa). Two of these studies reported a general increase in θfc while the overall effect was not reported for the other two. One of these three studies reported a general increase in the water content at permanent wilting point (θpwp; -1.5 MPa), while the overall effect was not presented in the other two. The plant available water content (θpaw), defined as the difference between θfc and θpwp, increased in the two studies where overall effects were presented.
Pore sizes in biochars range over at least five orders of magnitude, from the sub-nanometer scale to pore diameters of the order of tens of micro-meters originating from partially preserved cellular structures Brewer , 2014. However, a large fraction of the pore volume in biochar consists of pores in the nanometer size range Downie , 2009. These pores will retain water at very low pressure potentials and therefore have the potential to increase θpwp upon biochar addition. It has been suggested that increases in θpaw may be due to the filling of existing soil macropores with biochar which would shift the pore size distribution from large pores that drain quickly to pores that can retain water at field capacity Liu , 2017. Biochar itself contains pores in the relevant size range (0.2—100 µm in diameter) to contribute to θpaw. Thus, inter-particle pores in biochar will also contribute to θpaw depending on the size distribution and shapes of the biochar particles and their effects on soil aggregation Burgeon , 2021. Since θfc is the sum of θpwp and θpaw, the same processes are the likely causes of the observed increases in θfc.
The effects on water retention were in most cases larger for coarse-textured soils. Biochar with large microporosity can fill the larger inter-particle soil pores present in sandy soils so that the pore size distribution shifts towards smaller pores which can retain water at the pressure potentials corresponding to field capacity Edeh , 2020Omondi , 2016Razzaghi , 2020Rabbi , 2021. Moreover, fine-textured soil has a larger water retention capacity at θfc so that the relative changes induced by biochar may be smaller Edeh , 2020. All seven meta-analyses included data on biochar production parameters (e.g. feedstock, pyrolysis temperature) and the chemical and physical properties of biochar. Generally, the influence of these parameters on the effects of biochar addition were minor with respect to soil water retention. Due to lack of data, the influence of the time between biochar application and measurements on the effects on water retention was not included. It is, however, clear from studies on century-old charcoal kiln sites that the properties of biochar and associated soil evolve over time Cheng , 2008Hardy , 2017.
One out of three meta-analyses showed an increase in saturated hydraulic conductivity following biochar addition, one showed a decrease, while the third study did not report overall effects. Saturated hydraulic conductivity is a function of the pore network properties, including connectivity of the macropores and pore bottle-necks Koestel , 2018. A few studies have quantified the effects of biochar addition on the connectivity of macropore networks using X-ray tomography (e.g. Yu & Lu (2019); Yan (2021)). These studies indicate that the connected macroporosity and the diameter of pore throats decrease in medium- to coarse-textured soils amended with biochar. However, the influence of soil texture on the effects of biochar on saturated hydraulic conductivity reported in the meta-analyses was not consistent.
Two meta-analyses reported measures of aggregate stability. Omondi (2016) included only studies that reported mean weight diameter (MWD) using wet sieving, while Ul Islam et al., 2021 included studies that reported soil aggregate stability as a percentage of water-stable aggregates (WSA), as well as MWD or gravimetric mean diameter (GMD) using either wet sieving or dry sieving. Both studies showed that aggregate stability increased with biochar addition. The reason proposed for this effect was the influence of the added biochar on aggregation processes. The effects on aggregate stability increased with the time between biochar application and measurements Islam , 2021 .
Only one meta-analysis focused on water use efficiency Gao , 2020. They showed that both plant water use efficiency defined as the ratio of plant or fruit biomass to water supply, and leaf water use efficiency defined as the ratio of carbon dioxide uptake by leaves to the loss of water through transpiration, increased with biochar addition. Most biochars are alkaline and may increase soil pH, at least for acidic soils, which leads to improved conditions for plant growth. Biochars also contain nutrients that may become available for plant uptake. Indeed, previous studies have shown that the addition of biochar to nutrient poor acidic soils improves yields Jeffery , 2017. Interestingly, in the meta-analysis of Gao et al. (2020), soil pH had different effects on plant and leaf water use efficiency. Leaf water use efficiency increased most for soils with pH less than 7, while plant water use efficiency increased most for soils with pH above 8. The reasons for these results could not be determined from their study. Medrano (2015) showed that leaf water use efficiency may not be well correlated with plant water use efficiency. The large variability in reported effects (-36–313%) can be mainly attributed to differences in soil pH, biochar properties and amounts of added biochar.
The effects of the studied variables were in many cases larger for higher application rates. Often, laboratory studies used much larger application rates (>50 t ha-1) than the field studies, probably for economic reasons. This may explain why effects usually were larger in laboratory or greenhouse experiments compared to field trials. Additionally, as pointed out by Rabbi (2021), mixing of biochar after field applications is challenging and may be another reason why effects were sometimes small or insignificant for field experiments. The majority of the studies included in the meta-analyses were short-term experiments (i.e. duration < 1 year). Future work should therefore focus on longer-term effects of biochar applications under realistic field conditions. This requires either long-term field experimentation, which is expensive, or the study of historic biochar sites. It is also impractical to study these effects for the almost infinite number of combinations of soils, biochars and climate that exists. The meta-analyses included in this study, which show large variations in effects for all the included variables, suggest that future work should also be directed towards finding biochars with specific properties (e.g. surface area, particle size) designed to improve soil physical properties under specific soil and climate conditions while maintaining or improving nutrient availability.
Other organic amendments, residue retention and mulching¶
Figure 3 shows that only a few meta-analyses have focused specifically on the effects of organic soil amendments or residue retention and mulching on soil properties relevant for water regulation functions. Instead these practices are often included in meta-analyses on conservation agriculture or tillage systems. In these studies, the effects of the treatments are combined. Furthermore, the influence of contrasting soils or climates have not been assessed.
Bai (2018) studied the effects of different organic amendments applied in long-term field experiments on soil physical and hydraulic properties. They found that aggregate stability increased with organic amendments and that this effect was largest for compost. However, this beneficial effect decreased with time. Not surprisingly, Bai (2018) also reported greater aggregate stability under organic farming systems compared with conventional agriculture. Xiong (2018) also included soil amendments applied to agricultural land in their global meta-analysis on soil conservation practices. They found that application of soil amendments reduced both surface runoff and soil erosion. However, they provided no information on the type of amendments and how they were incorporated into soil. Gravuer (2019) analysed effects of organic amendments (manure, biosolids and compost) applied to arid, semi-arid and Mediterranean rangelands. They found increased water contents at field capacity and reduced surface runoff. Additional benefits were increased soil organic carbon contents and above-ground net primary productivity, while trade-offs were increased CO2 emissions, increased soil lead concentrations and increased losses of N and P in surface runoff.
Mulching means adding (or retaining) material on the soil surface without incorporation Kader , 2017. In this review, we focus on organic mulches, but synthetic materials are also used. The most extreme example of mulching with artificial materials is plastic mulching, which has been shown to increase crop water efficiency under drought Yu , 2021. The use of organic materials may have a number of beneficial effects on soil quality and the environment and it is, therefore, one important part of conservation agriculture. Mulching is typically carried out to limit soil evaporation, reduce soil runoff and erosion but it also affects, among other things, nutrient cycling, weed infestations and soil carbon storage Ranaivoson , 2017. Mulching was included as one driver in four meta-analyses that studied effects on soil hydraulic functions. These meta-analyses showed positive effects on the rather limited number of hydraulic properties included. Three meta-analyses (one for agricultural land Xiong , 2018, one for non-perennial crops Ranaivoson , 2017 and one focusing only on tree crops Liu , 2021 included effects of mulching on surface runoff. They all showed reduced surface runoff. The study for non-perennial crops also showed reduced soil evaporation and increased infiltration. These effects are already well-established in the scientific literature and in line with the intentions of mulching Kader , 2017. The meta-analysis by Li (2019) focused on effects of different tillage practices. Here we include the comparison between residue retention and no residue retention in no-till systems. Li (2019) showed that residue retention led to a decrease in bulk density, an increase in total porosity and an increase in plant available water while it did not have significant effects on saturated hydraulic conductivity. They attributed this to increased accumulation of organic material on the soil surface, which leads to increased biological activity and soil aggregation.
Irrigation¶
Several recent meta-analyses have investigated the impacts of so-called deficit irrigation on water use efficiency and/or yields of a range of agricultural crops Cheng , 2021Adu , 2018Yu , 2020Cheng , 2021Qin , 2016. The objective of this approach to irrigation scheduling is to reduce water use without significantly impacting yields by limiting the supply of water during periods of the growing season when it is less critical for crop growth. One of these meta-analyses Cheng , 2021 also synthesized the results of studies investigating the effects of partial root zone irrigation on water use efficiency and crop yields. This method also has the objective of saving water without impacting yields, but in this case by alternately supplying water to only one part of the root zone at each irrigation. These meta-analyses show that although these irrigation scheduling methods either have mostly neutral or sometimes positive effects on crop water use efficiency (see Figure 3), crop yields are significantly smaller compared to full irrigation for almost all crops and soil types. This implies that crop yields may in some cases be reduced less than water consumption, although these water savings may not compensate farmers for their yield losses. Another way to conserve high quality fresh water resources is to make use of brackish or saline water for irrigation. In their meta-analysis, Cheng (2021) showed how the decreases in water productivity, irrigation use efficiency and crop yields as a result of the use of salty irrigation water (see Figure 3) depends on crop type, irrigation methods, climates and soil type.
Based on the results of a meta-analysis, Qin (2016) suggested that eliminating over-optimal (excess) irrigation by more efficient irrigation scheduling would improve the water use efficiency of citrus by 30% and yields by 20%. Du (2018) showed that so-called aerated irrigation increases water use efficiency and yields of cereals and vegetables by ca. 20%, presumably by eliminating the development of anoxic conditions following irrigation.
Simulation models¶
Meta-analyses based on data from long-term field experiments have enabled us to identify some broad overall impacts of soil and crop management practices on water regulation and other related soil properties and processes. Ideally, we would like to achieve a more holistic and mechanistic understanding of these effects to support the development of climate change adaptation strategies by enabling predictions of rates of change and future impacts of changes in climate, land use and management. Soil-crop simulation models are potentially powerful tools in this respect Stöckle & Kemanian, 2020Robertson , 2015Nendel , 2018Jones , 2017 because they can “add value” to long-term field experiments. For example, soil-crop models can be used to identify plausible explanations of the observed treatment effects by filling in gaps in the data with respect to variables that were not measured. Once calibrated and validated against field data, they can also be used predictively, to extrapolate in time Constantin , 2012 and also in space (e.g. Hoffmann (2016); Coucheney (2018)) and to perform scenario analyses. Soil-crop models should prove especially useful as tools to evaluate the trade-offs and synergies associated with alternative management practices, particularly if they can be linked to economic analyses (e.g. Parvin (2022)).
Most studies of this kind to date have focused on the effects of rather simple management adaptations to climate variability or climate change, such as changes in dates of crop planting and harvest (e.g. Elliott (2018) ; Constantin (2019)). In contrast, there are surprisingly few examples of the use of soil-crop models to evaluate the effects management practices on properties and processes relevant for climate change adaptation (e.g. Nendel (2018)). However, in one recent example, Tribouillois (2018) used the STICS model to assess the impacts of introducing cover crops on the water and greenhouse gas balance at five different sites in southern France in rain-fed and irrigated cropping systems in present and future climates (2007 – 2052). They concluded that cover crops could potentially reduce greenhouse gas emissions from French agriculture from 5% to 9% by increasing soil carbon sequestration. However, cover crops also reduced drainage by ca. 20 mm/year and thereby potentially also groundwater recharge. Similarly, Meyer (2020) calibrated the STICS model Brisson , 2003 against data from a field experiment and then used the model to demonstrate that cover crops reduced annual drainage by 20-60 mm compared to plots under bare soil and would significantly reduce soil water contents for the next cash crop by, on average 20-50 mm, and by up to 80 mm under dry spring conditions. Scenario simulations with the calibrated model showed that mechanical destruction of the cover crop in late autumn and retaining the residues as mulch, would represent a good compromise between the multiple ecosystem services the cover crop provides during the fallow period, whilst avoiding the negative impacts on soil water availability for the next cash crop. Finally, Launay (2021) used the STICS model to assess the potential for cover cropping to enhance carbon storage in soil and reduce N20 emissions in France. The modelling was used to quantify trade-offs with respect to crop yields and water consumption, with the latter increasing by 13%, which was projected to increase the irrigation demand by ca. 80%. In another example, Cresswell (1992) simulated the effects of conventional and no-tillage systems and the presence of surface seals and plough pans on the generation of surface runoff with a hydrological model (SWIM). They concluded that substantial changes in the soil water balance required major alterations in soil structure such as the development of surface crusts or plough pans. The model simulations, which were based on field-measured hydraulic properties and thus purely predictive, also suggested that surface runoff would be greater from conventionally-tilled land under high intensity or prolonged rain, but that soil evaporation rates are larger from no-tilled soil.
Model predictions are inevitably associated with errors arising from uncertainties in both model parameter values and process descriptions Wallach & Thorburn, 2017Beven, 2006Wallach , 2021Tao , 2018. Soil-crop models require comprehensive input data to support model parameterization, calibration and validation. Unfortunately, experimental data is inevitably scarce in relation to the uncertainty in model parameters. In calibrating models in such cases, parameter errors may compensate for model deficiencies leading to non-unique solutions (‘equifinality’, Beven (2006)). In addition, most data sets are too short to properly reflect contrasting weather conditions, which would be fundamental to constrain model predictions in a future climate. Parameter uncertainty is not always considered in soil-crop model applications. For example, Seidel (2018) reported that nearly half the respondents to a questionnaire only used “trial and error” calibration. In such cases, even though a model appears to match the field data satisfactorily, it may be doing so for the wrong reasons, which means that model predictions, for example for a future climate, could be seriously in error Bellocchi , 2011He , 2017Kersebaum , 2007Kersebaum , 2015. Multi-model comparisons have shown that differing calibration strategies among model users increases the variation of results among models that arises from contrasting process descriptions even when they are calibrated on the same data (Wallach (2021)). This suggests the need for systematic and agreed protocols for calibration and validation of models in space and time.
Thus, despite their great potential and increasingly widespread use, few sufficiently comprehensive and critical tests have been carried out, so the validation status of many commonly used soil-crop models is unclear. In their recent review of crop modelling, Silva & Giller (2020) suggested that too little effort has been focused on model testing and model improvements. Specifically with respect to soil hydrology, the situation is reasonably clear-cut, since many crop models employ out-of-date process descriptions that are known to be inadequate. Brilli (2017) highlighted the fact that these deficiencies in current generation soil-crop models often result in poor soil water balance simulations. This is probably because most soil-crop models were originally developed by teams of crop scientists who focused primarily on the description of plant physiology and crop growth with little, if any, input from soil physicists. This has resulted in an imbalance in model process descriptions, in which the importance of accurate descriptions of soil hydrological processes has often been underestimated. Empirical (phenomenological) approaches are commonly used to describe crop growth. This is understandable because the underlying processes are extremely complex and are still not easily amenable to mechanistic descriptions Boote , 2013Wu , 2016. However, most soil-crop models also use empirical models to describe water flow and storage in the soil (e.g. tipping bucket or reservoir models; Brilli (2017)) even though physics-based approaches based on Richard’s equation are not difficult to parameterize, they are applicable to a wider range of hydrological conditions (e.g. shallow groundwater) and they also perform better (e.g. Diekkrüger (1995); Kröbel (2010); Guest (2017)).
Similarly, many widely used soil-crop models represent crop water uptake and transpiration with overly simplified empirical models, even though suitable physics-based models are now available Lier , 2013Lier , 2008Javaux , 2013Couvreur , 2012Sulis , 2019. These two issues are to some extent linked, as physics-based approaches to water uptake by plant roots require information on soil hydraulic functions that are not needed in tipping bucket or reservoir models. Model benchmarking studies Heinen, 2014Santos , 2017Willigen , 2012 and comparative model tests against field data Cai , 2018Cai , 2018 have demonstrated the errors that can be introduced by inadequate empirical descriptions of water uptake by crop roots, particularly those that do not account for compensatory mechanisms (e.g. Jarvis (2011)). The simplest physics-based models contain no more parameters than empirical models and they are also easier to estimate since they have a stronger physical basis Javaux , 2013Willigen , 2012. Thus, in principle, the predictive use of these models should also be more robust than empirical models.
It is frequently observed that plant roots may bypass soil layers that are compacted or otherwise hard to penetrate by preferentially growing through larger soil macropores (e.g. Hatano (1988); Stewart (1999); White & Kirkegaard (2010); Gaiser (2013); Kautz (2013)). The amounts of water and nutrients extracted by roots whilst growing through macropores may be limited by poor contact with the surrounding soil (White & Kirkegaard (2010) ). Nevertheless, the ability of plants to by-pass compacted or other otherwise strong soil layers enables them to exploit water and nutrients stored in deeper soil layers with more favourable physical conditions (Colombi (2018)). Most soil-crop models do not recognize the control of root growth by soil structure and soil strength Stöckle & Kemanian, 2020Wang & Smith, 2004 and the few exceptions mostly do so in a quite simplistic way, by making use of bulk density as a proxy for soil strength Robertson , 2015Maharjan , 2018. Better descriptions of root growth are therefore needed in soil-crop models to support simulations of the effects of soil management (e.g. tillage and traffic compaction) on the crop water supply. Novel approaches to modelling the effects of soil constraints on plant root growth have been developed in recent years, some of which could be exploited in more widely used soil-crop models. For example, Gaiser (2013) proposed a macroscopic approach that takes into account the effects of soil macropores on root growth in the soil profile. More recently, Landl (2017) developed a root architecture model that accounts for the effects of soil strength on the growth and elongation of plant root systems, and thus the ability of plant roots to exploit pathways of least resistance through the soil such as soil macropores. De Moraes et al (2018) coupled this root architecture model with functions relating root penetration to soil water status and soil strength as well as a soil hydrological model based on Richards’ equation including a sink term for water uptake by plant roots. Model predictions were compared with field data for soybean in Brazil and scenario simulations were run to illustrate the effects of a compacted soil layer on root growth and crop water uptake patterns.
One fundamental limitation of many soil-crop models is that they lack explicit descriptions of important management practices in conservation agriculture (Brilli, et al., 2017). For example, with some exceptions (e.g. Dilla (2018)), most soil-crop models cannot simulate spatially-distributed cropping systems such as inter-cropping or agro-forestry. Similarly, most models cannot explicitly simulate the dynamic effects of tillage loosening and traffic compaction (Brilli (2017)) as they assume that soil hydraulic properties are constant. A few modelling studies have employed empirical descriptions of within-year variations of soil physical and hydraulic properties induced by tillage loosening and subsequent consolidation (e.g. Strudley (2008); Maharjan (2018)). However, no existing soil-crop model can simulate changes in soil physical and hydraulic properties over longer time-scales such as decades or centuries, driven by climate and soil and crop management and mediated by soil biological agents and processes. This is the case even though the individual processes driving soil structure dynamics are reasonably well understood Meurer , 2020Meurer , 2020Young , 1998and its importance for soil hydrological processes has long been recognized (e.g. Cresswell (1992); Connolly (1998)). There may be several reasons for this. Firstly, the web of interacting processes governing structure dynamics (Figure 1) is complex and difficult to quantify. Secondly, changes of soil hydraulic properties over time are rarely monitored in field experiments at the required time-scales necessary for model calibration and testing, most probably because these properties have always been considered to be static. In this respect, long-term changes arising from land use and climate change may also be hard to distinguish and disentangle from the changes in soil properties due to short-term management effects and within-year variations in weather. Existing soil-crop models can be used to quantify the effects of soil management on soil structure and hydrological processes at any given moment of time by running scenario simulations with contrasting soil physical and hydraulic properties (e.g. Cresswell (1992)). However, such an approach cannot give any insights into the relevant time-scales of change. This is an important limitation when using existing soil-crop models to assess the implications of conservation agriculture for soil hydrological functioning and climate change adaptation. Some progress has recently been made towards the development of dynamic modelling approaches. For example, Meurer , 2020Meurer , 2020 proposed a simple concept for a modelling framework to account for soil structure dynamics arising from biological processes and agents such as root growth, earthworm bioturbation and soil organic matter turnover. Once incorporated into existing soil-crop models, this approach would enable simulation of the effects of long-term variations in pore size distribution, porosity and hydraulic functions on water flow, storage, crop water uptake and growth. Another limitation of soil-crop models relevant to climate change adaptation is that some plant traits that are important for water regulation are treated as constants, whereas plants may adapt and acclimatize, with key traits responding plastically to changes in environmental conditions (e.g. Nicotra & Davidson (2010); Vincent (2020); Jarvis (2021)).
Multi-model ensembles have been proposed as a way to account for errors and uncertainties in model process descriptions. Such an approach may help to improve the reliability of model predictions compared with single model applications (e.g. Wallach (2018)). However, ensemble modelling will not help if the consensus view is wrong. It should be more important to focus on replacing inadequate model descriptions by demonstrably better alternatives.
Conclusions¶
A large number of meta-analyses have been published in recent years on the impacts of soil and crop management practices on soil properties and processes and the various ecosystem services and functions delivered by soil. In this report, we have synthesized these analyses with respect to the water regulation functions that are relevant for climate change adaptation in Europe. This synthesis has revealed a considerable degree of consensus concerning the effects of soil and crop management practices, despite the fact that meta-analyses cannot easily account for differences in experimental conditions among individual source studies, not least because many primary studies do not report all details of the experimental treatments. This overview has also identified several important knowledge gaps, particularly related to the effects of soil management on crop root growth and transpiration. Thus, conclusions related to the impacts of management on the crop water supply are necessarily based on inferences derived from proxy variables such as available water capacity and infiltration capacity.
Meta-analyses have demonstrated that the use of organic amendments and the adoption of cropping systems and practices that maintain, as far as possible, “continuous living cover” both result in significant beneficial effects for the water regulation function of soils, arising from the additional carbon inputs to soil and the stimulation of biological processes. These effects are clearly related to improvements in soil structure, both in terms of stable aggregation at the micro-scale and enhanced bio-porosity, both of which reduce surface runoff and increase infiltration. Meta-analyses show that amendment of soils with biochar generally increases aggregate stability, reduces bulk density, increases porosity and improves the plant available water capacity, particularly for coarse-textured soils.
One potentially negative consequence of management practices that maintain “continuous living cover” is a reduction in soil water storage and groundwater recharge that, in most cases, will likely outweigh any increases in soil water storage capacity due to carbon sequestration. This may be problematic in dry climates, although there is no clear evidence to suggest that yields of the main crop are affected. With respect to environmental quality, no other significant trade-offs are known, while some important synergies have been identified, in particular reductions in nitrate leaching to groundwater and greenhouse gas emissions. It can be noted here that the meta-analyses reviewed here have not distinguished between types of cover crop systems with respect to timings of sowing and termination, even though this may strongly influence the subsequent main crop and the environment Meyer , 2020.
The evidence from meta-analyses to support the idea that reductions in tillage intensity improve crop water supply is limited and contradictory. The effects of no-till on SOM stocks and thus the capacity of the soil to store plant-available water appear to be minimal. In contrast, the amelioration of soil structure that occurs under RT and NT practices may improve infiltration capacity and reduce surface runoff, despite the increases in bulk density that are commonly reported, although the evidence for this is contradictory. Some significant trade-offs with RT and NT systems have also been identified. For example, yield penalties incurred under NT and increased weed pressure and/or increased herbicide use and thus leaching risks, especially in wetter and colder climates, constitute a barrier to adoption by farmers. Furthermore, greenhouse gas emissions are generally larger under NT, while leaching losses to groundwater of both nitrate and pesticides may also increase. Although we might expect losses of agro-chemicals in surface runoff to generally decrease under RT and NT, thereby compensating for greater leaching losses, this does not always appear to be the case. Reduced tillage intensity in the temporal sense (i.e. “occasional” tillage) may help to ameliorate some of the negative effects of no-till systems, whilst retaining some of the advantages.
In combination with field experiments, and despite uncertainties concerning the descriptions of soil hydrological processes in some instances, soil-crop simulation models should have an important role to play in resolving remaining knowledge gaps and evaluating trade-offs and synergies with respect to the impacts of management practices. It should be important to focus more efforts on improving the hydrological components of soil-crop models and their capability to describe the effects of a wider range of soil and crop management practices.
- IPCC. (2021). Climate Change 2021: The Physical Science Basis. Contribution of Working Group I to the Sixth Assessment Report of the Intergovernmental Panel on Climate Change [Masson-Delmotte, V., P. Zhai, A. Pirani, S.L. Connors, C. Péan, S. Berger, N. Caud, Y. Chen, L. Goldfarb, M.I. Gomis, M. Huang, K. Leitzell, E. Lonnoy, J.B.R. Matthews, T.K. Maycock, T. Waterfield, O. Yelekçi, R. Yu, and B. Zhou (eds.)] [Techreport].
- Palm, C., Blanco-Canqui, H., DeClerck, F., Gatere, L., & Grace, P. (2014). Conservation agriculture and ecosystem services: An overview. Agriculture, Ecosystems & Environment, 187, 87–105. 10.1016/j.agee.2013.10.010
- Schreefel, L., Schulte, R. P. O., Boer, I. J. M., Schrijver, A. P., & Zanten, H. H. E. (2020). Regenerative agriculture – the soil is the base. Global Food Security, 26, 100404. 10.1016/j.gfs.2020.100404
- Beillouin, D., Ben-Ari, T., & Makowski, D. (2019). Evidence map of crop diversification strategies at the global scale. Environmental Research Letters, 14(12), 123001. 10.1088/1748-9326/ab4449
- Beillouin, D., Ben-Ari, T., & Makowski, D. (2019). Evidence map of crop diversification strategies at the global scale. Environmental Research Letters, 14(12), 123001. 10.1088/1748-9326/ab4449